Translate this page into:
Dysbiosis of Gut Microbiota in Patients Undergoing Cardiac Surgery
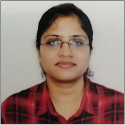
*Corresponding author: Manita Paneri, Centre for Interdisciplinary Biomedical Research, Adesh University, Bathinda, Punjab, India. manitaprashant@outlook.com
-
Received: ,
Accepted: ,
How to cite this article: Paneri M, Sevta P. Dysbiosis of gut microbiota in patients undergoing cardiac surgery. Glob J Med Pharm Biomed Update 2022;17:13.
Abstract
The diversity of bacteria, viruses, eukaryotic organisms, and archaea that live in the gastrointestinal tract and have coevolved with the host over thousands of years to establish a complex and advantageous relationship is referred to as the “gut microbiota” or “gut microbiome.” The numerous and diverse gut microbiota play an important role mostly in the betterment of human health by facilitating the breakdown of food to release nutrients that would otherwise be inaccessible to the host, encouraging host cells proliferation and differentiation, defending the host against pathogen colonization, and activating or modulating the immune system. The gut microbial community plays an important role in protecting the host against pathogenic microbes, modulating immunity, and regulating metabolic processes and is even regarded as an endocrine organ. The term “dysbiosis” refers to the imbalance of the gut bacterial microbiota, which has been linked to several disorders such as cardiovascular diseases, obesity, irritable bowel syndrome, colorectal cancer, and sepsis. During cardiac surgery, intestinal ischemia-reperfusion causes an inflammatory response throughout the body. Since the intestines are an organ that is vulnerable to ischemia, germs and endotoxins can move between organs. As a consequence, the gut leads to sepsis after surgery. Next-generation sequencing technologies have enabled analysis of a large number of microorganisms. Metagenomics can be used to study intestinal microbiome diversity and dysbiosis, as well as its relationship to health and disease. Sequence-based and multifunctional metagenomics will also provide insights that will lead to greater knowledge than it is ever been of the structural and functional microbiomes.
Keywords
Gut microbiota
Dysbiosis
Next-generation sequencing
Cardiac surgery
Metabolites
INTRODUCTION
The gut microbiota is a varied community of microbes that live in the human gastrointestinal system.[1-3] Antibiotic use and surgery, for example, can change the dynamics of the gut microbiota.[4,5,8] This is especially important in the hospital context, where the prevalence of bacteria that are resistant to antibiotics and the usage of antibiotics is higher.[6-8]
The human gastrointestinal system is the ecological niche in the body, where bacteria are most prevalent. Since each person’s gut microbiota is unique, there is no single ideal composition.[6] According to Sender et al., in 2016, human microbiota consists of 1014 microbial cells, with an around 1:1 ratio of microbial to human cells.[9]
Elie Metchnikoff was the first scientist to suggest that it was possible to modify the gut microbiome by replacing bad bacteria with good bacteria. He postulated that Bulgarian farmers, who lived in harsh conditions and poverty, had an anti-aging effect due to the consumption of fermented milk that contained Lactobacillus bulgaricus.[10] Jacbbsson et al., in 2014, found that phylum level comparison between vaginally delivered and cesarean section delivered children revealed a greater population of Bacteroidetes than Firmicutes.[11,12]
To carry out metabolic and immunological tasks as efficiently as possible, a balanced host-microorganism relationship must be maintained.[13,14] Diet, proper hygiene, and pharmaceutical medicines are only a few examples of the variables that might have a big influence on gut microbiota.[4,15-17] The effects of several environmental stimuli, which may have an impact on the microbiome’s composition and number, can have a significant impact on the gut microbiota.
Recent research suggests a direct association between infectious diseases and pathological alterations in the composition of the gut microbiome.[18]
To identify the microorganisms, present in samples, a technique called metagenomic analysis that targets the 16S rRNA gene has been developed.[19-21] It has made it possible for scientists to conduct sequence-based analyses of species that they had previously believed were inaccessible, including obligate anaerobes and other bacteria.[19] Using 16S rRNA deep sequencing, Ojima et al. (2016) were the first to assess the change in gut microbiota sequentially during the acute period in intensive care unit patients.
GUT MICROBIOTA: COMPOSITION
The phrase “gut microbiota” or “gut microbiome” refers to the diversity of bacteria, viruses, eukaryotic microbes, and archaea that inhabit the GI tract and have coevolved with the host over thousands of years to develop a complex and beneficial interaction [Figure 1 and Table 1].[3,16,22]
![Dominant Phyla of gut microbiota.[23]](/content/119/2022/17/1/img/GJMPBU-17-13-g001.png)
- Dominant Phyla of gut microbiota.[23]
Phyla | Features | Representative genera |
---|---|---|
Firmicutes | obligate and facultative anaerobes Mostly Gram-positive can form endospores that are resistant to O2, UV radiation, desiccation, extreme temperature, and chemical disinfectant | Ruminococcus Enterococcus Lactobacillus Clostridium |
Bacteroidetes | Aerobic and non-aerobic, non-spore forming, Gram-negative bacilli | Bacteroides |
Actinobacteria | Aerobic and non-aerobic, Gram-positive bacteria, high G+C content in their DNA | Bifidobacterium |
Proteobacteria | Facultative anaerobes, wide range of Gram-negative bacteria | Enterobacteriaceae |
GUT MICROBIOTA: BENEFITS
Metabolic function
The human gut microbiota aids in the breakdown of undigested carbohydrates, such as big polysaccharides such as cellulose, hemicellulose, and pectin, as well as unabsorbed sugar and alcohol, which are converted into a variety of short-chain fatty acids (butyrate, propionate, and acetate).[25,26] Short-chain fatty acids (SCFAs) are metabolite byproducts of bacteria and they have shown positive outcomes in the treatment of numerous disorders such as obesity, diabetes, inflammatory bowel diseases, colorectal cancer, and neurological problems.[25,27,28]
Analysis of a public metagenome database revealed that 92% of individuals contained at least one of the rare Bacteroides species capable of digesting xyloglucans, which humans are unable to digest.[30]
In addition, the gut microbiota aids in the absorption of ions such as magnesium, iron, and calcium as well as the production of vitamins (Escherichia coli create Vitamin K).[6,30]
Trophic function
The trophic function of the human gut can be significantly influenced by short-chain fatty acids (SCFs) generated by certain gut microbiomes.[26] The proliferation and differentiation of the epithelial cells are stimulated by the three primary SCFs (butyrate, propionate, and acetate).[31] Butyrate is an energy currency for colonocytes and also has anticancer properties. It can also inhibit histone deacetylase, thus aiding in the regulation of gene expression.[32,33] Propionate provides energy to the epithelial cells and also takes part in gluconeogenesis.[34] In the human body, acetate is utilized for lipogenesis and cholesterol metabolism.[31,35]
Protective function
To prevent invasion and subsequent infection by different harmful microorganisms, the human gut microbiome acts as the first line of defense alongside the host defense systems. By preventing harmful bacteria from attaching to or entering the human gut, the resident gut microbiota helps the body resist colonization. Lactobacillus acidophilus fights for available nutrients with other pathogens and also produces bacteriocin to impede the competitor’s growth.[36]
In addition to directly outcompeting pathogens for nutrients, intestinal microbes also increase mucus production and intestinal epithelial integrity, modify bile salts to make them toxic to other microorganisms, and can produce antibacterial peptides.[37]
By encouraging epithelial cells to produce antibacterial agents and boosting humoral defenses against invasive pathogens, gut bacteria aid in the development of resistance to enteric pathogens.[5]
Piewngam et al. (2018) demonstrated that Staphylococcus aureus colonization is eliminated when probiotic Bacillus bacteria are consumed. This is the 1st-time interference that has been accomplished by suppressing a pathogen’s signaling mechanism.[38]
Citrobacter rodentium is competitively excluded from the gut by Bacteroides thetaiotaomicron because it consumes the carbohydrates required by the pathogen.[39]
Endocrine function
According to Brown and Hazen (2015), gut microbial symbionts assemble to create a crucial endocrine organ that transforms environmental nutritional cues into hormone-like signals that influence both normal physiology and chronic illness in the human host, while being largely ignored in the science of endocrinology.[40] Recent research reveals that several gut microbial metabolites are detected by certain host receptor systems to slow the onset of cardiovascular disease (CVD).[41] In actuality, proatherogenic circulatory substances are produced as a result of gut microbial metabolism of food components and influence CVD risk through a meta-organismal endocrine axis. A crucial new concern in the field of cardiovascular medicine is whether or not pharmaceutical therapies at the level of the gut microbial endocrine organ will lower the risk of CVD.[40]
The hypothesis that hormone-like metabolites from the gut can operate at remote places in the central nervous system is now being supported by a developing understanding of how gut bacteria affect neurotransmission in the brain. When taken as a whole, these factors place the gut microbial endocrine organ at the center of both the synthesis of its own hormones and the modulation of the signaling tone of host hormones.[40,42]
COMPLICATIONS AND RISK FACTORS FOR CARDIAC SURGERY
Complications such as fever, delirium, bloodstream infection, hemodynamic instability,[41] pseudopodia,[18] surgical site infection, ventilator-associated pneumonia, and systemic inflammation might be possible after cardiac surgery.[41,43-45] In the hospital environment, patients over or under-aged, undergoing surgery, immunocompromised, receiving mechanical ventilation are at higher risk of getting antibiotic resistance bacteria. Following cardiac surgery, antibiotics and systemic inflammation can disrupt the gut microbiome.[4,19,45]
The consequences of cardiac surgery and the healing process are strongly impacted by the high incidence of postoperative gastrointestinal problems. Diarrhea, bloating, gastrointestinal bleeding, and other abdominal problems following cardiac surgery are probably caused by alterations in the gut flora.[46]
REASONS FOR BACTERIAL DYSBIOSIS AFTER CARDIAC SURGERY
Antibiotics
The main site of colonization for pathogens is the gastrointestinal tract. The selection forces of intensive care treatment led the gut to almost completely collapse ecologically. As a result, it is possible to see that a condition of gut-derived sepsis emerges at the end of a severe illness.[47]
By altering the gut microbiota’s antibiotic resistance, antibiotic therapy can make people more vulnerable to nosocomial and opportunistic infections. In addition, the microbiota’s physiological processes including the synthesis of vitamins and the metabolism of carbohydrates may be lost. Inflammatory bowel disease and higher mortality after stem cell transplantation have both been linked to decreased gut microbial diversity. Pre-antibiotic commensal microbiota inhibits the expansion of oxygen-tolerant bacterial species. Compositional analyses of colonic microbial populations in humans after antibiotic treatment showed that loss of obligate anaerobes frequently results in the expansion of Gammaproteobacteria and enterococci.[48-50]
Maekawa et al., 2020 in their research study, found that after cardiac surgery patients had significantly lower bacterial count due to the administration of antibiotics, gut microbiota composition also changed and the pH of fecal matter was also higher than it was before surgery.
The butyric acid concentration was higher in pre-operative samples (8.7 ± 5.9 µmol/g of feces) than first post-operative and second post-operative sample (4.9 ± 3.19 µmol/g of feces; and 5.2 ± 5.59 µmol/g of feces). They found that in the post-operative sample, Enterobacteriaceae, Enterococcus, and Staphylococcus counts were higher. Patients who developed pseudopsia after cardiac surgery have been seen at a higher level of Staphylococcus, Enterobacteriaceae, and Pseudomonas counts. Lactobacillus are major components of gut microbiota, but post-operative Lactobacillus counts were found to be significantly low.[18]
Maekawa et al., in 2020, suggested that post-operative mental disorders may be related to considerably higher postoperative fecal pH. After cardiac surgery, SCFA-secreting bacteria like Lactobacillus reduced, suggesting that the microbiome was harmed, resulting in leaky gut syndrome.[18]
Zaborin et al., in 2014, mentioned that when organisms are exposed to opioids, commensalism between Candida albicans and bacteria can be interrupted, raising the idea that the level of host stress may contribute to the community’s transition toward a more pathogenic state.[47] Zaborin et al., in 2014, first demonstrated that during protracted critical illness, 2-member communities of C. albicans plus bacteria can predominate over the healthy gut microbiota.
Zheng et al. (2017) demonstrated that following cardiac surgery, levels of several bacteria, including Oscillibacter, Anaerotruncus, Alistipes, and Clostridium difficile (CD), were higher in their study. The suppression of the production of inflammatory cytokines is associated with these microorganisms. The prevalence of Eubacterium and butyrate-producing bacteria has dramatically decreased.[46]
BLOOD STREAM INFECTION
Adamik et al. (2017) in their research study found that long-term cardiopulmonary bypass (CPB) can increase intestinal permeability that allowed microorganisms and their endotoxins to pass from the gut into the bloodstream.[51]
Wang et al., (2019) in their research study found that patients with the early post-operative bloodstream infections associated with the Enterobacteriaceae family had considerably longer CPB times as well as lower early and late survival rates when compared to those without postoperative bloodstream infections.[52]
According to Ding et al., (2020) Gram-negative bacteria found in the gut flora are responsible for the early bloodstream infections that develop in individuals receiving CPB. Growing data support the notion of gut flora transfer. During cardiac surgery with CPB, intestinal ischemiareperfusion will trigger a systemic inflammatory response and might result in intestinal flora translocation.[42] According to Dickson (2016), alteration in the gut flora and metabolites can trigger systemic inflammatory responses and the body’s immunological response in severely sick individuals, which can result in sepsis. Intestinal dysbacteriosis following CPB may be a contributing factor to inflammation and an imbalanced immune system’s anti-inflammatory response.[53]
In healthy individuals, the genus Staphylococcus of facultative anaerobic bacteria typically colonizes the nares and epidermis, but in pre-operative cardiac patients, the carriage is linked to an increased risk for postoperative surgical site infection and bacteremia.[54]
Ruminococcus is a resident microbe that also produces SCFAs; nevertheless, in specific circumstances, they may cause inflammation and bloodstream infection.[55]
CLOSTRIDIUM DIFFICILE INFECTION (CDI)
The most well-known pathogen linked to outbreaks after antibiotic-mediated disruption to the microbiome is CD.[48] CD is an anaerobic, Gram-positive bacillus. CD is the most common cause of hospital-acquired diarrhea, leading to increased morbidity and mortality in surgical patients. CDI is the third most common major infection after pneumonia and bloodstream infections following cardiac surgery.[56]
Rzucidło-Hymczak et al. (2021) mentioned that patients who had cardiac surgery and had high glucose levels or stress hyperglycemia during the initial post-operative period were more likely to acquire CDI. Patients having CDI in this research exhibited higher post-operative lactate levels than the control group. In addition, individuals with CDI had decreased lactate clearance, which worsened hyperlactatemia by impairing lactate clearance.[56]
B. thetaiotaomicron has been shown to cause a competitive exclusion of C. rodentium. It may also cleave sialic acid moieties from mucin and create high levels of succinate, which can boost the colonization of CD.[39]
COUNTER STRATEGIES AGAINST COLONIZATION RESISTANCE
The host is shielded by the gut microbiota from enteric diseases brought on by invading microbes or native pathobiont expansion. Colonization resistance is the term used to describe the strategies that the microbiota uses to thwart the development of pathogens. Pathogens have created defense mechanisms to increase their population and pathogenicity.[57]
The gut epithelium mucosal layers, which are known as mucin, act as protective barriers rich in sugar components. These sugar molecules are metabolized by saccharolytic members of the gut bacterial community. Pathogenic bacteria can also utilize these available nutrients for the proliferation of their growth. The gut lumen can release large amounts of nitrogen and carbon from ethanolamine. As a pathogen-specific nutrient, it may be used by a variety of pathogenic bacterial species. Most pathogens have developed strategies to consume alternative nutrients or to exploit the widely available nutritional resources more effectively to combat commensal nutrient competition.
Pathogenic bacteria also employ such tactics to gain an advantage over commensals in terms of proliferation. The majority of pathogenic gut microorganisms create toxic substances and other virulent components that cause gut inflammation. Diarrhea or intestinal inflammation brought on by a pathogen significantly changes the composition of the gut microbial population. Increased Proteobacteria and reduced Firmicutes would result in inflammatory responses, creating a porous gut that would allow the penetration and transfer of microbial-related metabolites.
The elimination of taxa including Blautia, Ruminococcus, Faecalibacterium, Pseudobutyrivibrio, and Subdoligranulum was a characteristic shared by all severely sick individuals demonstrated by Lankelma et al., 2017.[49]
The primary features of the intestine microbiological alterations following the surgery were an elevation in Enterococcus, a decline in alpha diversity, and anaerobes. It is generally known that cardiovascular patients undergoing general anesthesia, whether or not patients were supported by CPB, would have surgical trauma and ischemic intestine reperfusion damage, which had been proven as causing intestinal microbes to become disturbed.[58]
Obligate anaerobes, such as Bacteroidetes or Firmicutes, comprise the majority of the commensal microbiota in the gut. These organisms cannot use nitrate (NO3) as an electron acceptor. NO3 reductase enzymes are expressed by pathogenic bacteria like E. coli, which may use them as an energy source to flourish.
An unfavorable inflammatory condition in the gut could be facilitated by the absence of Faecalibacterium prausnitzii, a bacteria recognized for its anti-inflammatory characteristics. Inflammation alters the physiological environment of the gut and the nutrient profiles that are available, which may cause commensal bacteria to be inhibited and pathogenic bacteria to proliferate. Inflammation also causes the expression of distinct metabolic pathways and virulence genes that are not present in commensals.
It is conceivable that disrupted gut bacterial colonization, most likely the loss of the core intestinal microbiota, serves as a risk factor and a clinical sign of heart failure.[59]
Pathogens need to use the metabolites released by the microbiota as both signals and nutrients to successfully colonize and invade a host.
NUTRIENT DEPLETION
Hypoxia, hypercapnia, the use of medications to reduce gastric secretion, the use of sedatives, and analgesics that impair gastrointestinal tract motility, parenteral, and enteral nutrition that deprive the microbiome of nutrients – for example, microbially accessed indigestible carbohydrates – and, of course, the use of antimicrobials are the main causes of dramatic changes in microbiota composition.[41]
Some microbes make better use of substances such as iron, and for that, some bacteria generate siderophores. As a defense mechanism, host cells release lipocalin-2, which can inhibit enterobactin (Ent), an E. coli siderophore, reducing iron uptake and thus commensal E. coli growth in the gut. Myeloperoxidase, a host-produced bactericidal enzyme, is effectively inhibited by Ent, a catecholate siderophore secreted by E. coli, according to research by Singh et al., 2015.[60]
Even when adequate intravenous nutrition is provided, dietary restriction causes the gut bacteria to lack macronutrients, which results in a relative decrease of Firmicutes and an increase of Proteobacteria and Bacteroidetes. In contrast to Firmicutes that dominate in an ecosystem having abundant nutrients, Proteobacteria have been found to thrive in conditions of relative starvation.[61]
BACTERIAL METABOLITES AND BACTERIOCIN
Amyloids and lipopolysaccharides, which the gut microbiota may produce in large amounts, may contribute to the production of pro-inflammatory cytokines such as tumor necrosis factor (TNF). TNF may serve as a suppressor of cardiovascular function by diminishing mitochondrial activity, disrupting calcium homeostasis, and weakening adrenergic signaling in cardiomyocytes.[42,62]
Reduced intestinal motility impacts the bacterial population’s supply of energy substrates, perhaps causing a bacterial shift since the bacterial metabolites, endotoxins, and food components are in the gut for a longer period of time.[59]
Increased amounts of circulating endotoxins and bacterial metabolites cause systemic inflammation and oxidative stress on trimethylamine N-oxide (TMAO), a metabolite that is produced in the gut and has been associated with the development of CVD.[63-66]
Pathogens can create toxins or inhibitors that can specifically target the normal gut flora. Vibrio cholerae can transfer toxic effectors to E. coli directly through its type VI secretion system. In addition, pathogenic Shigella strains and Salmonella have been observed to produce bacteriocin. Bacteriocins likely can contribute to the successful colonization of the intestine by pathogenic bacteria by targeting specific commensals and thereby distorting barrier maintenance, immune surveillance, and/or gut metabolism to facilitate their invasion.[39]
The risk of atherosclerosis and thrombosis may be influenced by TMAO produced by the gut flora. These results are consistent with Haghikia et al., 2018 discovery that gut microbiota reduction can stop the growth of Ly6Chi monocytes.[64]
Functional genes involved in tryptophan production were also discovered to be less prevalent in post-operative individuals. The necessary and crucial amino acid tryptophan serves several key functions. Intestinal 3-indolepropionic acid influences the expression of pro- and anti-inflammatory genes in intestinal epithelial cells as well as tight junction protein expression. Furthermore, a deficiency in tryptophan has been associated with several diseases. As a result, it is possible that following cardiac surgery, the gut microbiota’s ability to produce tryptophan was diminished, which led to gastrointestinal complications.
Although Anaerococcus can metabolize amino acids and peptones and produce short-chain fatty acids (SCFAs), including butyric acid, they can also be linked to persistent wounds, skin and soft-tissue infections, and other diseases.
Chernevskaya et al. (2021) assessed the metabolic activity of microbiota and found that taurine level was high in the infectious group. It is well known that the intestinal microbiota causes taurine to disintegrate into hydrogen sulfide. High hydrogen sulfide concentration can inhibit the activity of cytochrome oxidases and, as a consequence, aerobic respiration, which is one of the main contributors to the pathogenicity of bacteria.[41] Chernevskaya et al., 2021, discovered that increased levels of TMAO were related to an increase in the ratio of Proteobacteria to Firmicutes.
Human opportunistic bacterial pathogen Pseudomonas aeruginosa induces nosocomial infection by binding to the host immune factor interferon with its outer membrane surface protein OprF.
PREVENTION
The pathophysiological process is greatly influenced by the gut microbiota, which may also be a promising early biomarker. There are significant taxonomic anomalies in the intestinal microbiota of patients associated with cardiovascular disorders. Microbiota-targeted therapeutics can enhance the efficacy of cardiac surgery.[41]
It may be possible to improve the outcome of surgeries by preventing a dysbiosis state ahead of surgery. It might be helpful to “characterize” a patient’s microbiota before the procedure. Utilizing cutting-edge probiotics may increase resistance to the effects of surgery and hospitalization on the microbiota.[67] Probiotics are beneficial colonies of microbes that are extracellularly administered and tend to substantially reestablish the skewed microbial diversity, hence reducing pathogenic issues in surgical and critically sick patients.[61]
“Synbiotics” is the term for the combination of prebiotics and probiotics, which are indigestible fibers that support probiotic proliferation and activity. By re-establishing intestinal permeability and reducing the intestinal inflammatory response, probiotics can preserve the health of the gut barrier. They also keep the native gut flora in balance.
CONCLUSION
Research on the gut microbiome is currently advancing, and this has yielded mechanistic insights into the interplay between commensals and pathogens. These insights may assist to establish alternative strategies for disease prevention and eradication.
Benefits from next-generation sequencing provide fascinating possibilities to identify the gut microbiome’s composition in a critically ill patient. Predicting a particular pathogen community’s behavior in the context of shifting the clinical course will be challenging.[47]
Probiotics supplementation and dietary changes to introduce beneficial bacteria can help modify the microbiota. Future research needs to be focused on nutrition and the intestinal barrier, which will be an advanced and promising area of study.
Studying the particular association and probable mechanism of fever of unknown origin with hemodynamic instability after CPB is of utmost necessity to explore the characteristics of intestinal microecology in patients who underwent cardiovascular surgery with CPB.
Declaration of patient consent
Patient’s consent not required as there are no patients in this study.
Financial support and sponsorship
Nil.
Conflicts of interest
There are no conflicts of interest.
References
- Gut microbiome and health: Mechanistic insights. Gut. 2022;71:1020-32.
- [CrossRef] [PubMed] [Google Scholar]
- The first microbial colonizers of the human gut: Composition, activities, and health implications of the infant gut microbiota. Microbiol Mol Biol Rev. 2017;81:e00036-17.
- [CrossRef] [PubMed] [Google Scholar]
- Microbiota in health and diseases. Signal Transduct Target Ther. 2022;7:135.
- [CrossRef] [PubMed] [Google Scholar]
- The intestinal microbiota: Antibiotics, colonization resistance, and enteric pathogens. Immunol Rev. 2017;279:90-105.
- [CrossRef] [PubMed] [Google Scholar]
- What is the healthy gut microbiota composition? A changing ecosystem across age, environment, diet, and diseases. Microorganisms. 2019;7:14.
- [CrossRef] [PubMed] [Google Scholar]
- Antibiotics and the human gut microbiome: Dysbioses and accumulation of resistances. Front Microbiol. 2016;6:1543.
- [CrossRef] [PubMed] [Google Scholar]
- Dysbiosis of intestinal microbiota in critically ill patients and risk of inhospital mortality. Am J Transl Res. 2021;13:1548-57.
- [Google Scholar]
- Revised estimates for the number of human and bacteria cells in the body. PLoS Biol. 2016;14:e1002533.
- [CrossRef] [PubMed] [Google Scholar]
- Gut microbiome: What we do and don't know. Nutr Clin Pract. 2015;30:734-46.
- [CrossRef] [PubMed] [Google Scholar]
- The mode of delivery affects the diversity and colonization pattern of the gut microbiota during the first year of infants' life: A systematic review. BMC Gastroenterol. 2016;16:86.
- [CrossRef] [PubMed] [Google Scholar]
- Decreased gut microbiota diversity, delayed Bacteroidetes colonisation and reduced Th1 responses in infants delivered by caesarean section. Gut. 2014;63:559-66.
- [CrossRef] [PubMed] [Google Scholar]
- Interaction between microbiota and immunity in health and disease. Cell Res. 2020;30:492-506.
- [CrossRef] [PubMed] [Google Scholar]
- Microbiome and pathogen interaction with the immune system. Poult Sci. 2020;99:1906-13.
- [CrossRef] [PubMed] [Google Scholar]
- Gut microbiota metabolism and interaction with food components. Int J Mol Sci. 2020;21:3688.
- [CrossRef] [PubMed] [Google Scholar]
- The athletic gut microbiota. J Int Soc Sports Nutr. 2020;17:24.
- [CrossRef] [PubMed] [Google Scholar]
- Diet and the microbiota-gut-brain axis: Sowing the seeds of good mental health. Adv Nutr. 2021;12:1239-85.
- [CrossRef] [PubMed] [Google Scholar]
- Association between postoperative changes in the gut microbiota and pseudopsia after cardiac surgery: Prospective observational study. BMC Surg. 2020;20:247.
- [CrossRef] [PubMed] [Google Scholar]
- Metagenomic analysis reveals dynamic changes of whole gut microbiota in the acute phase of intensive care unit patients. Dig Dis Sci. 2016;61:1628-34.
- [CrossRef] [PubMed] [Google Scholar]
- Application of metagenomics in the human gut microbiome. World J Gastroenterol. 2015;21:803-14.
- [CrossRef] [PubMed] [Google Scholar]
- Metagenomic analysis of gut microbiome and resistome of diarrheal fecal samples from Kolkata, India, reveals the core and variable microbiota including signatures of microbial dark matter. Gut Pathog. 2020;12:32.
- [CrossRef] [PubMed] [Google Scholar]
- Introduction to the human gut microbiota. Biochem J. 2017;474:1823-36.
- [CrossRef] [PubMed] [Google Scholar]
- Human microbiomes and their roles in dysbiosis, common diseases, and novel therapeutic approaches. Front Microbiol. 2015;6:1050.
- [CrossRef] [PubMed] [Google Scholar]
- Role of the normal gut microbiota. World J Gastroenterol. 2015;21:8787-803.
- [CrossRef] [PubMed] [Google Scholar]
- Gut microbiota functions: Metabolism of nutrients and other food components. Eur J Nutr. 2018;57:1-24.
- [CrossRef] [PubMed] [Google Scholar]
- Carbohydrates great and small, from dietary fiber to sialic acids: How glycans influence the gut microbiome and affect human health. Gut Microbes. 2021;13:1-18.
- [CrossRef] [PubMed] [Google Scholar]
- Human gut microbiota in health and selected cancers. Int J Mol Sci. 2021;22:13440.
- [CrossRef] [PubMed] [Google Scholar]
- The role of the gut microbiome in diabetes and obesity-related kidney disease. Int J Mol Sci. 2021;22:9641.
- [CrossRef] [PubMed] [Google Scholar]
- A discrete genetic locus confers xyloglucan metabolism in select human gut Bacteroidetes. Nature. 2014;506:498-502.
- [CrossRef] [PubMed] [Google Scholar]
- Gut microbiota and iron: The crucial actors in health and disease. Pharmaceuticals (Basel). 2018;11:98.
- [CrossRef] [PubMed] [Google Scholar]
- Intestinal short chain fatty acids and their link with diet and human health. Front Microbiol. 2016;7:185.
- [CrossRef] [PubMed] [Google Scholar]
- The neuropharmacology of butyrate: The bread and butter of the microbiota-gut-brain axis? Neurochem Int. 2016;99:110-32.
- [CrossRef] [PubMed] [Google Scholar]
- Butyrate and the intestinal epithelium: Modulation of proliferation and inflammation in homeostasis and disease. Cells. 2021;10:1775.
- [CrossRef] [PubMed] [Google Scholar]
- Supplementation of a propionate-producing consortium improves markers of insulin resistance in an in vitro model of gut-liver axis. Am J Physiol Endocrinol Metab. 2020;318:E742-9.
- [CrossRef] [PubMed] [Google Scholar]
- Role of gut microbiota-generated short-chain fatty acids in metabolic and cardiovascular health. Curr Nutr Rep. 2018;7:198-206.
- [CrossRef] [PubMed] [Google Scholar]
- The anti-apoptotic and anti-inflammatory effect of Lactobacillus acidophilus on Shigella sonnei and Vibrio cholerae interaction with intestinal epithelial cells: A comparison between invasive and non-invasive bacteria. PLoS One. 2018;13:e0196941.
- [CrossRef] [PubMed] [Google Scholar]
- Antimicrobial peptides and the enteric mucus layer act in concert to protect the intestinal mucosa. Gut Microbes. 2014;5:761-5.
- [CrossRef] [PubMed] [Google Scholar]
- Pathogen elimination by probiotic Bacillus via signalling interference. Nature. 2018;562:532-7.
- [CrossRef] [PubMed] [Google Scholar]
- When pathogenic bacteria meet the intestinal microbiota. Philos Trans R Soc Lond B Biol Sci. 2016;371:20150504.
- [CrossRef] [PubMed] [Google Scholar]
- The gut microbial endocrine organ: Bacterially derived signals driving cardiometabolic diseases. Annu Rev Med. 2015;66:343-59.
- [CrossRef] [PubMed] [Google Scholar]
- Gut microbiota as early predictor of infectious complications before cardiac surgery: A prospective pilot study. J Pers Med. 2021;11:1113.
- [CrossRef] [PubMed] [Google Scholar]
- Clinical multi-omics study on the gut microbiota in critically Ill patients after cardiovascular surgery combined with cardiopulmonary bypass with or without sepsis (MUL-GMCSCPB study): A prospective study protocol. Front Med (Lausanne). 2020;7:269.
- [CrossRef] [PubMed] [Google Scholar]
- Surgical site infections in cardiac surgery. Crit Care Clin. 2020;36:581-92.
- [CrossRef] [PubMed] [Google Scholar]
- Ventilator-associated pneumonia after cardiac surgery: A meta-analysis and systematic review. J Thorac Cardiovasc Surg. 2014;148:3148-55.e1-5.
- [CrossRef] [PubMed] [Google Scholar]
- Prevalence and clinical impact of systemic inflammatory reaction after cardiac surgery. J Cardiothorac Vasc Anesth. 2019;33:1682-90.
- [CrossRef] [PubMed] [Google Scholar]
- Clinical parameters and gut microbiome changes before and after surgery in thoracic aortic dissection in patients with gastrointestinal complications. Sci Rep. 2017;7:15228.
- [CrossRef] [PubMed] [Google Scholar]
- Membership and behavior of ultra-low-diversity pathogen communities present in the gut of humans during prolonged critical illness. mBio. 2014;5:e01361-14.
- [CrossRef] [PubMed] [Google Scholar]
- Resurrecting the intestinal microbiota to combat antibiotic-resistant pathogens. Science. 2016;352:535-8.
- [CrossRef] [PubMed] [Google Scholar]
- Critically ill patients demonstrate large interpersonal variation in intestinal microbiota dysregulation: A pilot study. Intens Care Med. 2017;43:59-68.
- [CrossRef] [PubMed] [Google Scholar]
- Antibiotics as deep modulators of gut microbiota: Between good and evil. Gut. 2016;65:1906-15.
- [CrossRef] [PubMed] [Google Scholar]
- Prolonged cardiopulmonary bypass is a risk factor for intestinal ischaemic damage and endotoxaemia. Heart Lung Circ. 2017;26:717-23.
- [CrossRef] [PubMed] [Google Scholar]
- Cardiopulmonary bypass time predicts early postoperative Enterobacteriaceae Bloodstream Infection. Ann Thorac Surg. 2019;107:1333-41.
- [CrossRef] [PubMed] [Google Scholar]
- Risk prediction for Staphylococcus aureus surgical site infection following cardiothoracic surgery: A secondary analysis of the V710-P003 trial. PLoS One. 2018;13:e0193445.
- [CrossRef] [PubMed] [Google Scholar]
- First case of bloodstream infection caused by Ruminococcus gnavus in an 85 year old man in China. Lab Med. 2022;53:e36-9.
- [CrossRef] [PubMed] [Google Scholar]
- Prognostic role of perioperative acid-base disturbances on the risk of Clostridioides difficile infection in patients undergoing cardiac surgery. PLoS One. 2021;16:e0248512.
- [CrossRef] [PubMed] [Google Scholar]
- Mechanism of the gut microbiota colonization resistance and enteric pathogen infection. Front Cell Infect Microbiol. 2021;11:716299.
- [CrossRef] [PubMed] [Google Scholar]
- Elevated systemic and intestinal inflammatory response are associated with gut microbiome disorder after cardiovascular surgery. Front Microbiol. 2021;12:686648.
- [CrossRef] [PubMed] [Google Scholar]
- Heart failure is associated with depletion of core intestinal microbiota. ESC Heart Fail. 2017;4:282-90.
- [CrossRef] [PubMed] [Google Scholar]
- Interplay between enterobactin, myeloperoxidase and lipocalin 2 regulates E. coli survival in the inflamed gut. Nat Commun. 2015;6:7113.
- [CrossRef] [PubMed] [Google Scholar]
- Gut microbiome, surgical complications and probiotics. Ann Gastroenterol. 2017;30:45-53.
- [CrossRef] [PubMed] [Google Scholar]
- The gut microbiota and Alzheimer's disease. J Alzheimers Dis. 2017;58:1-15.
- [CrossRef] [PubMed] [Google Scholar]
- Levels of trimethylamine N-oxide remain elevated long term after left ventricular assist device and heart transplantation and are independent from measures of inflammation and gut dysbiosis. Circ Heart Fail. 2021;14:e007909.
- [CrossRef] [PubMed] [Google Scholar]
- Gut microbiota-dependent trimethylamine N-oxide predicts risk of cardiovascular events in patients with stroke and is related to proinflammatory monocytes. Arterioscler Thromb Vasc Biol. 2018;38:2225-35.
- [CrossRef] [PubMed] [Google Scholar]
- Relationships between gut microbiota, plasma metabolites, and metabolic syndrome traits in the METSIM cohort. Genome Biol. 2017;18:70.
- [CrossRef] [PubMed] [Google Scholar]
- Uncovering the trimethylamine-producing bacteria of the human gut microbiota. Microbiome. 2017;5:54.
- [CrossRef] [PubMed] [Google Scholar]
- Marked changes in gut microbiota in cardio-surgical intensive care patients: A longitudinal cohort study. Front Cell Infect Microbiol. 2020;9:467.
- [CrossRef] [PubMed] [Google Scholar]